Energy Impact Partners: A primer on the next three decades of the energy transition - Part 2.
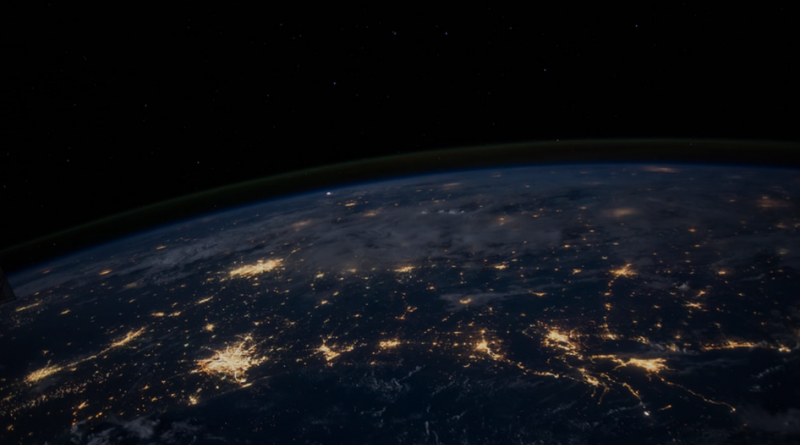
Energy Impact Partners research lead, Andy Lubershane, releases a five-part series examining technologies needed to fully decarbonize the energy system, relying on as few technology “moonshots” as possible.
This article is the second of a five-part series examining the technology we’ll need to fully decarbonize our energy system, relying on as few technology “moonshots” as possible.
I. The Electric Future We Need
II. Two Storage Technology Revolutions
If the movie “The Graduate” were produced today, the most famous lines of dialogue would be:
Mr. McGuire: I want to say two words to you. Just two words.
Benjamin: Yes, sir.
Mr. McGuire: Are you listening?
Benjamin: Yes, I am.
Mr. McGuire: Energy storage
Benjamin: Exactly how do you mean?
Mr. McGuire: There’s a great future in energy storage. Think about it. Will you think about it?
Like plastics — the “great future” technology of the original film — energy storage is what we think of in the venture capital world as “hard tech”. That doesn’t just mean it’s hard to find a winning solution, though it is — very hard. It also means that energy storage is one of those things that software can’t “eat”. Energy storage is a chemistry and physics problem that no amount of coding or business acumen can overcome. Blockchain won’t help us with this one…(or, in my opinion, with anything else…but that’s another barrel of monkeys).
Also like plastics, energy storage will take multiple forms — at least two forms, to be specific, though probably a dozen or so more. The reason is that many of our energy storage needs can already be satisfied by the leading battery technology of our time: Lithium-ion. Lithium-ion batteries are great for consumer electronics, and have proven adequate — though by no means stellar — for two other important functions: They’ve enabled the very-early-adoption of electric vehicles (EVs); and they’ve begun to compete with natural gas turbines to provide very short-term peaking capacity and ‘ancillary service’ needs on the electrical grid.
However, the current trajectory of Lithium-ion battery technology, on its own, does not appear up to the task of solving two critical energy storage challenges:
1. Storage for truly ubiquitous electric vehicles
2. Storage for weekly, monthly, & seasonal fluctuations in energy supply & demand
Lithium-ion: The unfortunately-not-quite-perfect storage solution
Lithium-ion is the reigning champion of the battery world, and it deserves the title. For about a century before Lithium-ion seized that mantle, we were mostly stuck with lead-acid batteries, which are relatively cheap, but can only be recharged a few hundred times — and only as long as you utilize no more than half of their storage capacity. (If lead-acid batteries are discharged below roughly 50% “depth”, their rechargeability suffers.) Lead-acid batteries are also too big and heavy to serve as the primary power source for a vehicle.
Enter Lithium-ion, in the early 1990s: more than five times as energy-dense as lead-acid; rechargeable thousands of times down to an 80% depth of discharge; and greater than 90% efficient from electricity input to electricity output. In many ways, Lithium-ion is the uber-battery. I’m guessing you probably have at least two of them within arm’s reach as you read this article. (In fact, I’d be willing to bet the e-ink you’re reading right now is powered by Lithium-ion.)
Initially, the problem with Lithium-ion was cost. As recently as 2010, twenty years after they were first commercialized, Lithium-ion batteries still cost about $1,000 per kilowatt-hour of energy that they were able to store. At that price, the battery pack for a 2020 Nissan Leaf would have cost about $60,000, on its own. Hence, despite its uber-battery status, Lithium-ion has been saddled by many naysayers; plenty of industry analysts — myself included, I’ll admit — were too bearish on the potential pace of progress.
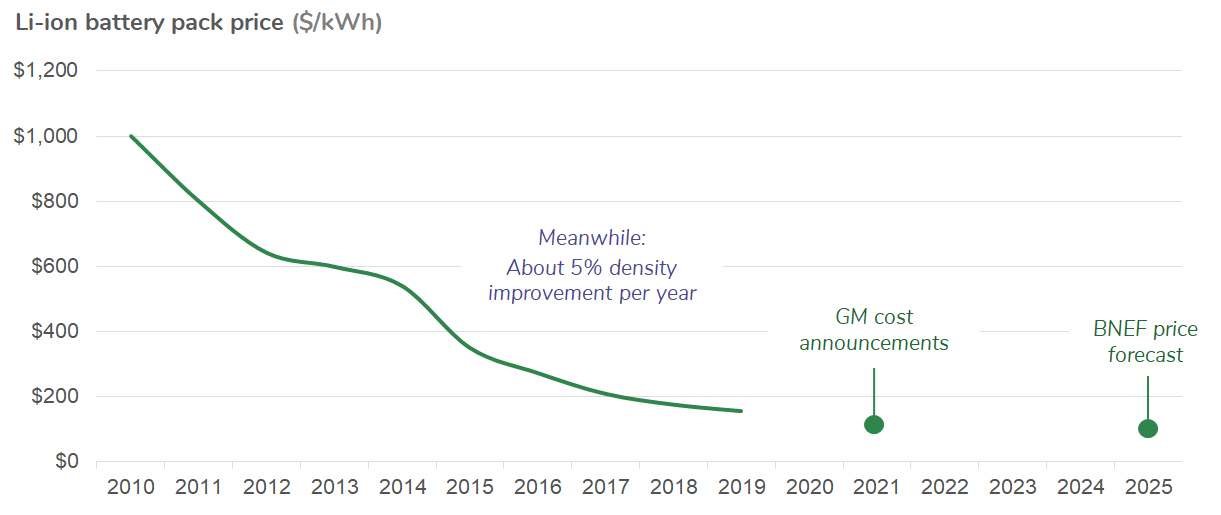
Just as for solar panels, the mega-scaling of the industry combined with the entry of new China-based manufacturers unlocked unforeseen cost reductions. Meanwhile, the underlying technology continued to make incremental advancements in energy-density and rechargeability.
And yet, amidst this tremendous progress, Lithium-ion has begun to display certain weaknesses — most notably in the supply chain. There’s certainly plenty of Lithium in the world — the estimated global resource could electrify the world’s light duty vehicle fleet 2.5 times over. But if EV demand scales up as quickly as we need it to, there will undoubtedly be short-term bottlenecks in supply. Current annual Lithium production couldn’t supply even 10% of annual vehicle sales if called upon in a pinch.
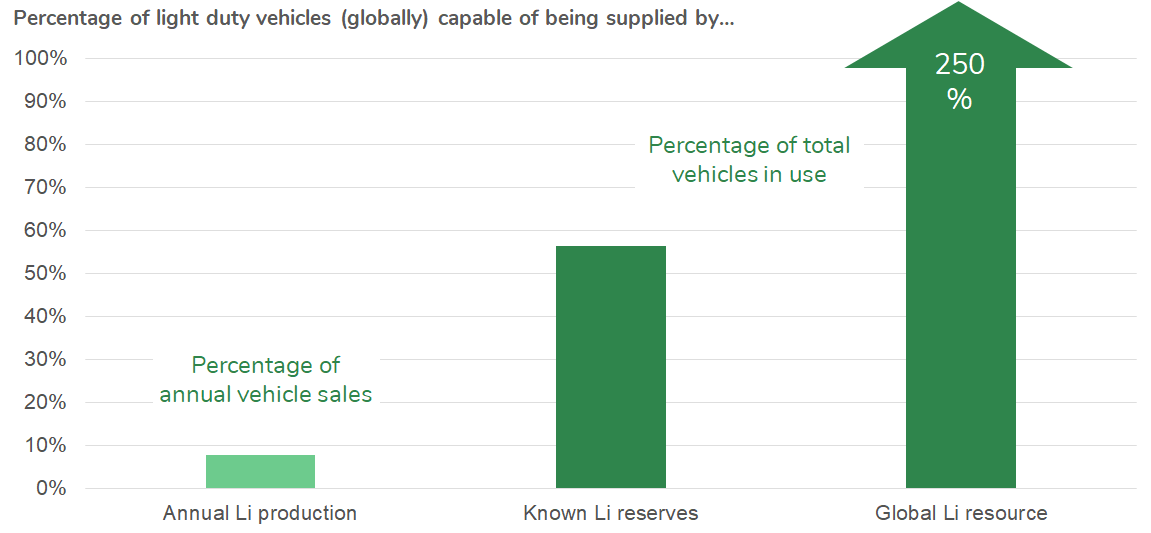
More importantly, Lithium-ion batteries rely on other elements as well. Lithium-ion is really a family of battery chemistries — all employing Lithium in the electrolyte, but with varying electrode materials. Many of the variants most commonly used in EVs rely on Cobalt, for which the supply chain is notoriously unethical. Almost all variants use a particular type of graphite, the production of which is smothering Chinese villages. Addressing these supply chain problems while continuing to make significant progress on cost and performance is a tall order.
Yet, even if Lithium-ion resolves its supply chain issues while sticking to the cost curve displayed above, it’s still unlikely to be the battery to end all batteries. To understand why, we need to take a close look at the two storage challenges it doesn’t appear able to solve.
Storage challenge #1: Truly ubiquitous electric vehicles
The pace and extent of electric vehicle adoption will depend on four interrelated technical factors (plus social factors and policy factors, of course). All four of the technical factors have to do with battery technology.
1. Battery cost
2. Battery density
3. Battery charging speed
4. Charging infrastructure
These four factors combine to determine whether the cost and functionality of an EV can be truly competitive with a good old-fashioned internal combustion engine (ICE). If you’ve driven an electric car, you know that the driving experience is phenomenal: quiet, smooth, sporty, etc. But you also know that “range anxiety” is real.
I recently became an EV driver; my wife and I bought a Nissan Leaf about a year ago (which I think of as “The People’s EV”). I love the Leaf, but I’ve already accumulated a handful of range-anxiety stories that would undoubtedly dissuade the typical driver from going electric.
The first key factor, battery cost, is the one that Lithium-ion is on track to overcome. My Leaf cost about $30,000 before applying any government incentives. Bloomberg’s battery price outlook anticipates a cost decline of about 50% by the mid-2020s. If that outlook is realized, Nissan could hypothetically sell the same Leaf, with the same margin, for $26,000, which is pretty comparable to the price of a new conventional hatchback with a similar look and feel. Comparing lifetime cost of ownership would probably make the EV the economic winner, owing to lower fuel and maintenance costs.
Depending on the weather and driver behavior, my Nissan Leaf gets about 100–150 miles of range from its 40 kilowatt-hour battery. (As an aside: Yikes, EVs lose a lot of range in cold weather!) If Lithium-ion batteries continue to gain energy-density at about 5% per year — as the CTO of Tesla once observed — then by 2025 Nissan could fit roughly 30% more battery in the same space, offering 130–195 miles of range on a single charge. Unfortunately, that’s still less than half of the ‘single tank’ range of a comparable ICE vehicle, which means stopping to refuel more than twice as often.
Range anxiety is partially a function of how much energy your battery can store in a single charge, but it’s also a function of how fast you can recharge that battery. ICE vehicle drivers safely assume they can refill their tanks almost everywhere in less than five minutes. Yet, given the current state of technology for batteries and EV charging stations, it’s impossible for an EV to even approximate that charging time. Most “fast-charging” stations today (known as “Level 3” chargers) can fill up a battery like my Leaf’s in about 45–60 minutes. To do so, they sacrifice longevity, as faster charging means significantly faster battery degradation. And woe be unto the unfortunate EV driver who pulls up to a lone fast-charging station, only to find another driver has just pulled in and begun their own 45–60 minute charge!
Of course, these range challenges will be exacerbated when trying to electrify larger, heavier vehicles — which tend to be more popular than Leaf-shaped cars among US consumers.
Therein lies the rub: Although Lithium-ion technology is on track to make EVs competitive economically, it’s not on track to make EVs competitive functionally. On extended trips away from home, they require drivers to stop more than twice as often, and each stop is sure to last at least ten times as long. These factors consign EVs to the role of “in-town” or “commuter” vehicles, which are only intended to charge slowly — most of the time at a house with a dedicated parking spot. In my view, that’s a critical constraint to mass market adoption.
This range problem is compounded by other technology and business model trends in the transport sector — most importantly the transition to fleets, and potentially even more so by the transition to autonomous fleets. Businesses built on vehicle fleets, whether or not they’re autonomous, are playing a utilization game. Time spent charging is time that’s not spent ferrying around customers. It doesn’t matter whether customers are paying by the ride (a la Uber & Lyft) or as a subscription; parking fleet vehicles at a charging station for 45 minutes every few hours is an inefficient use of capital. For autonomous vehicles — which will be essentially mini data centers plastered with sensors on wheels — maximizing utilization will be an even more urgent imperative.
Don’t get me wrong: we’re still in the very early days of EV adoption in most major markets. More Level 3 (and even Level 2) public charging stations will help encourage the next tranche of early adopters to make the leap from ICE to EV. However, the current generation of EV batteries and fast chargers are not fast enough to get us anywhere near 100% electrification. It’s hard to say exactly, but I’m skeptical they can even get us to 50%, given the preferences demonstrated to date by US consumers.
There are essentially two possible solutions to this core problem, and both are just beginning to progress from laboratories to pilot projects. The first is ultra-fast-charging technology.
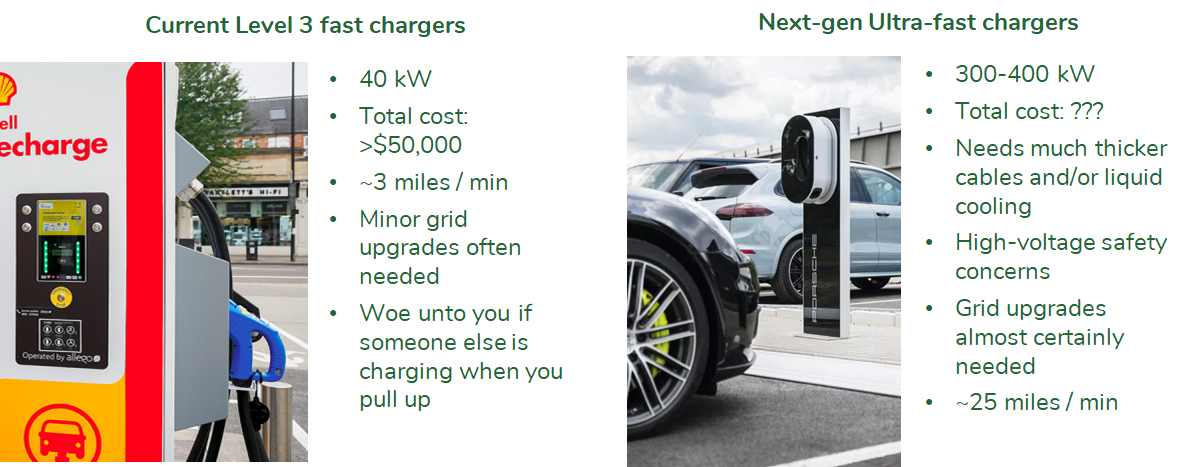
Ultra-fast chargers — designed to deliver 300–400 kilowatts — are capable of charging at about 10 times the rate of current fast chargers. That goes a long way towards solving the EV range problem. However, the laws of physics and common sense dictate that these chargers will be a lot more expensive to install. While charging, they draw about as much power from the grid as a whole neighborhood! More importantly, Lithium-ion batteries have already been shown to degrade much faster when regularly charged on the current generation of 40–50 kilowatt fast chargers. Hence, a transition to ultra-fast chargers as a regular mode of charging will need to be accompanied by a change in technology that limits the damage from such high voltage levels.
An alternative option is battery swapping technology, which unfortunately left a bad taste in investors’ mouths after the first attempt by an early pioneer ended poorly. But the goals of battery swapping remain tantalizing: avoid the morass of electrical grid constraints and battery degradation issues that ultra-fast charging introduces. Instead, a swapping system would charge batteries as much as possible at an ideal pace for both the batteries themselves, and for the power system. When a vehicle is running low on energy, it would simply pull up to a swapping station, and exchange its spent battery for a fresh one. Hypothetically, this swap could be accomplished almost as quickly as a stop at a gas pump.
The challenges for battery swapping are threefold. First, swapping only works if enough vehicles have standardized on a single battery form factor and swapping-ready design. Second, it means buying extra batteries that will, at times, be sitting ‘idle’ outside of a vehicle. Third, there’s the obvious technical difficulty of quickly swapping more than a thousand pounds of batteries in and out of a car.
This is certainly a daunting combination of business model and technological hurdles. It’s one that I hope clever entrepreneurs can overcome.
The path forward
In order to achieve widespread vehicle electrification, we’re going to need vehicles that can compete on both cost and functionality with ICE vehicles, and that means a combined revolution in battery technology and charging technology. Just one or the other won’t do; we need the whole package: batteries with about twice the density of today’s Lithium-ion, and with either: a) much greater resilience to ultra-fast charging, or b) an even lower cost profile to accommodate the ‘excess’ battery capacity required for swapping systems.
Just for fun, here’s a sample of the technology categories and companies pursuing this revolution, today.
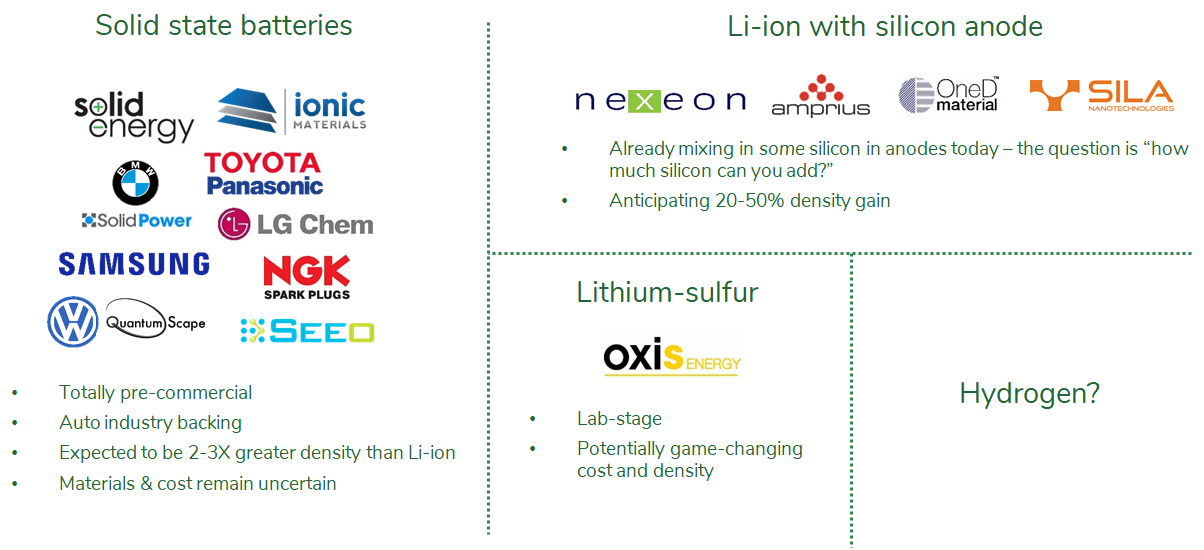
Storage challenge #2: Seasonal storage for the grid
The dramatically falling cost of Lithium-ion batteries has had ramifications beyond the world of EVs. It has also enabled stationary battery storage to become a feasible option for balancing the supply and demand of electricity on the power grid.
Electricity is unique among commodities in that consumption must be precisely balanced by production in real time. Otherwise, the lights go out. Lithium-ion batteries have started to be deployed cost-effectively to provide that balancing function for second-to-second fluctuations on the grid (a service known in the US as “frequency regulation”). But in most places, batteries are still struggling to compete with natural gas turbines to serve as the go-to grid-balancer for even a few hours at a time.
Fortunately, batteries are on track to become more competitive in short-term “peaking capacity” roles as costs continue to fall and more intermittent wind and solar generation is added to the system. In regions with relatively high solar energy penetration, such as California and the Southwest, Lithium-ion systems are starting to win contracts to provide peaking capacity during a relatively short window: four hours in the early evening as the sun sets and household electricity demand picks up.
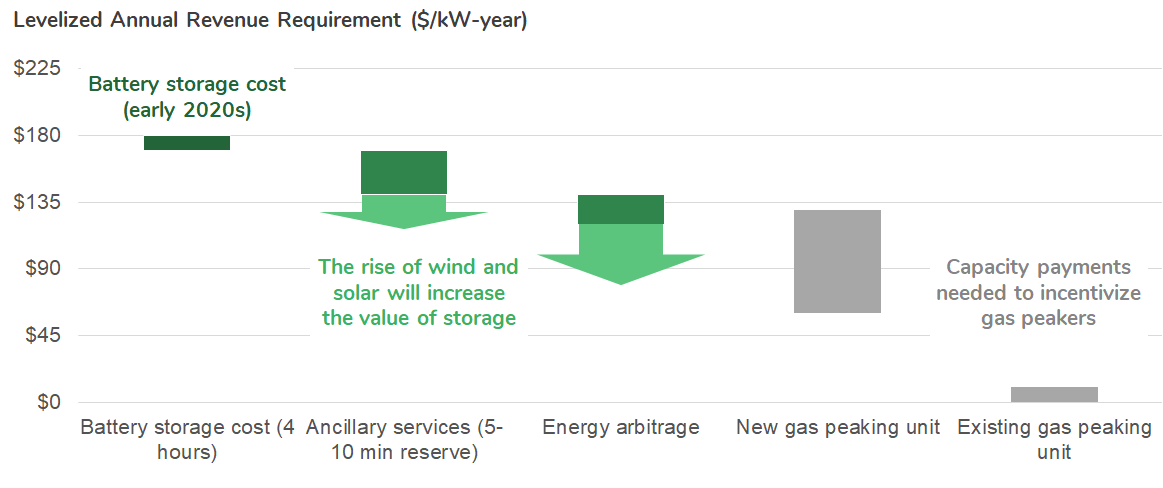
However, Lithium-ion batteries and other commercially available grid storage technology options (most notably, Flow batteries) all struggle to cost-effectively address what’s known as “longer-duration” storage needs. Asking current-generation batteries to provide energy for even 8–12 hours at a time starts to become very expensive. Yet, as the grid penetration of wind and solar increases beyond 50% (a very rough heuristic), 8–12 hour imbalances will begin to look quaint.
What emerges is the need for truly seasonal energy storage to address the inherent seasonal fluctuations in solar and wind power. A typical solar farm in North Carolina produces nearly 50% more energy in July than in January; and a wind farm in Oklahoma produces 70% more in January than July. Of course, demand for electricity fluctuates seasonally, too. It currently peaks in most regions in the summer, when air conditioners are running full blast. If, however, we electrify space heating, then electricity demand in cold climate regions could increase by more than 5-fold during the winter months.
To illustrate the problem, here’s a representative graph showing hourly energy demand on the electrical grid managed by the Midcontinent Independent System Operator (which spans much of the Midwest US, from the Dakotas down to Arkansas and Louisiana). Actually, this graph shows a year’s-worth of what’s called “net load”, which is energy demand minus the contribution of wind, solar, and nuclear power production — all zero carbon energy sources that grid operators are unable to switch on and off at will.
The graph shows a hypothetical scenario in which production from those three sources, plus existing nuclear power, is equal to 100% of total energy demand over the course of the year. (Actually, make that 105% of total annual energy demand — because once we add storage to this scenario there will be some ‘round-trip losses’ with each cycle of charging and discharging.) To begin with, let’s take a look at this system with no storage added:
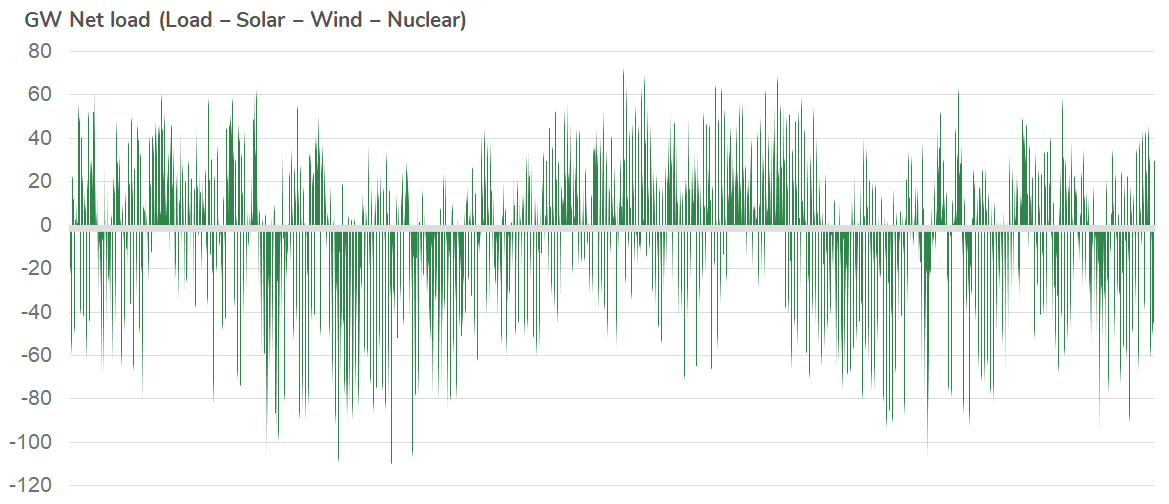
Upward spikes represent times when demand exceeds the supply of clean energy, and downward spikes below zero represent times when clean energy supply exceeds demand. Without storage, this system is clearly not going to work!
Here’s that same system, but with enough storage to balance all of the intra-day fluctuations in supply and demand. I’ve overlaid it on the first graph for illustrative purposes.
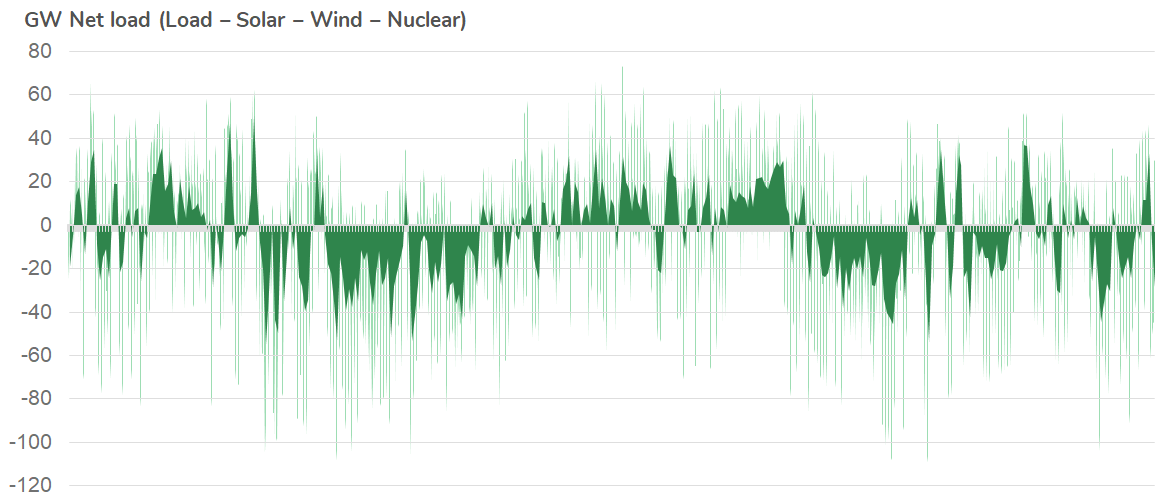
With about 275 gigawatt-hours of storage — the equivalent storage capacity of about 4 million EVs — all of the daily spikes are gone. This is the duty cycle that current storage technologies, like Lithium-ion batteries — are most well-suited to provide. But, you can see that major imbalances remain. So, here’s the same system, but with enough storage to balance all intra-month variability.
The seasonal storage problem is now clear as day. In order to balance this lingering seasonal mismatch in supply and demand, you’d need about 20 Terawatt-hours of storage capacity — equal to the batteries in roughly 350 million EVs. Now that might start to challenge the Lithium supply chain…
Fortunately, we won’t require the same rigorous performance requirements from this enormous amount of storage that we require from EV batteries. We don’t need seasonal storage to be super dense. We don’t need it to undergo 65 mile-per-hour crash tests. Perhaps most importantly, we don’t need it to be highly rechargeable; as the chart above illustrates, a long-duration storage system will only be called on for two very long charge/discharge cycles per year.
What we do need from seasonal storage, unfortunately, is a cost profile that appears…frankly, bonkers, relative to Lithium-ion batteries. While Lithium-ion battery prices are expected to drop a little bit below $100 per kilowatt-hour, we need seasonal storage to be at most half of that price — preferably, less than a tenth — if the cost of the energy transition is going to remain socially and politically acceptable.
This category of storage represents a significant gap in our ability to complete the clean energy transition. There is very little on the drawing board, with a few hopeful exceptions. Fortunately, we have time. Even if we achieve the aggressive wind and solar deployment pathway illustrated in the introduction to this series, seasonal storage will not become strictly necessary for balancing the grid until the final stretch — probably not until the late 2030s or early 2040s. That’s partly because natural gas can continue to fill in the gaps as a seasonal balancing fuel until we need to push the last 20–30% of carbon out of the system. But it’s also partly because seasonal storage isn’t the only solution to our seasonal imbalance problem. The other solution is to simply build more renewables.
Take another look at the annual ‘net load’ charts above. They’re actually illustrating a super high-end estimate of the amount of seasonal storage we might need. That’s because they present a scenario in which total clean energy generation is equal to only 105% of total electricity demand (remember, 100% plus a little extra to account for storage losses).
In reality, up to a point, we’ll want to keep building more renewables well beyond that level. Yes, those ‘extra’ solar and wind farms will produce too much electricity when it’s sunny and windy; in fact, they’ll produce so much excess energy that some of it will need to be “curtailed” (industry-speak for “wasted”). But they’ll also produce some electricity during the periods when there would otherwise be a shortfall — notably the spring and fall, when it’s neither especially sunny nor especially windy.
Wasting clean energy sounds bad, I’ll admit. But it’s likely to be the economical thing to do. If half of the energy from any given wind or solar farm is wasted, that means the cost of the useful energy it produces will need to be doubled. As long as that double-priced solar or wind energy is less expensive than seasonal storage, it will make sense to build more of it.
Of course, this strategy won’t work forever. The cost of additional wind and solar farms will increase as we need to locate them in incrementally worse locations; plus, each additional plant will also ‘waste’ more energy than the last. So, at some point we’ll need seasonal storage; but, it’s probably going to be the last piece of the puzzle.
To sum up: the current generation of Lithium-ion batteries are wonderful, but they’re not the only batteries we’ll ever need. They can only take us so far down the road to electrification and decarbonization. To get to the end of that road, we need two very different revolutions in storage technology. One, in the near term, needs to yield batteries that are a little cheaper, a lot denser, and more tolerant of fast-charging. Alongside these batteries, we also need to figure out how to deploy a national network of charging infrastructure that approximates the performance of our current network of gas stations. The other revolution, in the long term, needs to yield a storage system that’s much, much cheaper…but doesn’t need to fit in a car, and will only be called upon to fully ‘cycle’ once or twice per year.
Next up: MORE RENEWABLE ENERGY!
31 March 2020
Climate Action